Attempts Towards the Buckyball-amino Acid (Baa) Acylation of the 5�-Phospho-2�-deoxyribocytidylribo-adenosine (pdCpA)
Subunit
T. Amanda
Strom and Andrew R. Barron*
Department of Chemistry, Department of Materials Science and
Nanoengineering, Rice University, Houston, TX 77005. Email: [email protected]
Graphical Abstract
Abstract: The irreversible adsorption of fullerene (C60) substituted amino acids to the hydrophobic resin bead surface during solid phase peptide synthesis leads to low yields. Due to challenge in preparing sufficient C60-substituted phenylalanine (Buckyball-amino acid, Baa) an alternative route to fullerene-substituted peptides was investigated. The first step is the synthesis of the amino acylated 5�-phospho-2�-deoxyribocytidylribo-adenosine subunit (pdCpA-Baa) prior to enzymatically ligating it to a truncated tRNA. However, despite the successful synthesis of the cyanomethyl ester, Fmoc-Baa-OCH2CN (1) no reaction is observed between the hydrophilic pdCpA and hydrophobic Baa even in the presence of cationic surfactant or in DMF solution. As an alternative method a N,N�-diisopropylcarbodiimide coupling route was investigated, which despite the presence of an appropriate m/z in the MALDI-MS did not lead to an isolable product. The successful coupling of a hydrophobic perfluorophenyl ester (Fmoc-Gly-OPfp) to pdCpA suggests that it is steric bulk rather than miscibility that precludes the Baa coupling.
Keywords: Acylation, Bucky
amino acid, C60, phospho-cytidine-phospho-adenosine, fullerene
Introduction
Prato and co-workers were the first to prepare fullero-amino acids and were also the first to use the synthetic amino acids in the preparation of fullero-peptides through solid phase peptide synthesis (SPPS).1,2 They recognized that the novel structure of C60 in conjunction with an amino acid moiety provided unique opportunities to interact with the biological world through both its shape and scale. Since that time, many others have investigated C60 amino acids and peptides for their membranotropic,3-5 antioxidant,6 and antimicrobial7 properties. C60 peptides have also shown promise as enzyme inhibitors including HIV-1 protease, nitric oxide synthase, and carbonic anhydrase.8-11 Currently, fullerene peptides are prepared by solid phase peptide synthesis (SPPS); however, this technique is inherently problematic for the incorporation of fullerene amino acids into a peptide sequence, because Fullerene amino acids, with a large hydrophobic surface, irreversibly adsorb onto the hydrophobic resin bead surface. The irreversible adsorption results in less recovery of the fullerene amino acid starting material from the three-to-five times excess required for SPPS. Given the challenge of synthesizing and purifying C60 amino acids at a large scale, a more efficient procedure is desirable.
Peptide biosynthesis offers a potential alternative to SPPS.
A cell produces peptides and proteins through the ribosome, which is a large
quaternary protein that reads the code within mRNA to make specific protein
sequences. The ribosome pieces together proteins based on the genetic
information translated from the messenger RNA (mRNA). The mRNA contains a three
base sequence (codon) that complementarily encodes for a specific amino acid
carried by the transfer RNA (tRNA). In addition to its amino acid cargo, the
tRNA�s second important part is its anticodon. The anticodon is recognized
through complementary hydrogen bonding of nitrogenous base pairs with the mRNA
and told to drop off its amino acid cargo to become the next link in the
growing peptide chain.12 It is possible to hijack the ribosome and
essentially trick it into making peptides with unnatural amino acids through
the use of these nonsense codons. This process became known as nonsense suppression.13
Hecht,14,15
Chamberlin,16 and Shultz17,18 were pioneers in the work
of nonsense suppression and site-directed mutagenesis. Hecht�s group
used a truncated suppressor tRNA, with the last two nucleotides cut off,
specifically the phospho-cytidine-phospho-adenosine subunit (pCpA).14,15
This methodology, utilizing the dinucleotide pCpA, was
synthetically simplified by Shultz with the substitution of deoxycytidine
rather than cytidine as one of the nucleoside subunits creating the hybrid
dinucleotide (pdCpA) without sacrificing function.17,18 The
amino acylated pdCpA (pdCpA-aa), is then enzymatically ligated to the truncated
tRNA and fed into the ribosome for peptide biosynthesis. Over 50 unnatural
amino acids have been added to the genetic code through this technique.11
Despite the laborious nature and ultimately
moderate yield of synthesizing pdCpA-aa, and then enzymatically ligating it to
a truncated tRNA, it remains a widely used method of incorporating unnatural
amino acids through the ribosome mediated pathway.19-23 In our ongoing
effort to find efficient ways to incorporate fullerene amino acids into peptide
sequences, we decided to explore this biosynthetic pathway with our previously
reported Buckyball-amino acid (Baa).24 Fullerene amino acids capable
of forming peptide bonds have been prepared by many methods.4,6,9
Our laboratory has utilized a Diels-Alder addition of trimethylsiloxybutadiene
to the fullerene cage to form a C60-ketone intermediate with a
convenient handle for functionalization (Scheme 1). A condensation reaction with
Fmoc-Phe(4-NH2)-OH (Fmoc = fluorenylmethyloxycarbonyl) yielded an
imine linkage to C60 which was subsequently reduced by a BH3∙THF
complex. The result is an extremely stable fullerene amino substituted
phenylalanine (Baa, Scheme 1).4,6,24
Scheme 1: Synthesis of fullerene amino acid Fmoc-Baa through Diels-Alder addition intermediate C60-ketone
Results and Discussion
The acylation of a hybrid
dinucleotide, pdCpA, with the fullerene amino acid, Baa was investigated. The
unprotected hybrid dinucleotide (pdCpA) and the fullerene amino acid (Baa) were
synthesized by reported literature methods.23,24 The next and final
step is amino-acylation of the 2� or 3� hydroxyl of the sugar ring. Previous
reports use an �activated� amino acid in the form of a cyanomethyl ester to
limit nitrogen acylation of the nitrogenous bases.18 The challenges
we are facing are two-fold: 1) pdCpA is extremely hydrophilic with three
negative charges, while Baa is hydrophobic, and 2) the fullerene moiety is
sterically hindering.
In our investigation two different approaches were used to bring the hydrophilic pdCpA and hydrophobic Baa into contact with each other. The first attempt utilized a cationic surfactant (cetrimonium chloride, CTACl) to exploit the inherent properties of each reactant through the formation of positively charged micelles in solution (Scheme 2).25 The goal was to sequester the negatively charged pdCpA on the surface of the micelle while the hydrophobic Baa resides on the interior of the micelle. The increased local concentration of each component around and in the micelle should lead to acylation of pdCpA. No products were ever observed by this synthesis method and, in fact, in most cases the only result was hydrolysis of the cyanomethyl ester (see Experimental). We next tried the traditional approach of solubilizing pdCpA in dimethylformamide (DMF) by cloaking the negative charges with tetrabutylammonium (TBA) ions through ion exchange.17,18 While both reactants were soluble in DMF, through this method no reaction products were observed after multiple attempts (Scheme 2).
In an attempt to achieve oxygen acylation in
high yield, several coupling strategies, including carbodiimides and
perfluorophenyl esters, were considered. In a scheme utilizing coupling
reagents the free amines must be protected (phosphates are shielded as TBA
salts), whereas in published methods nitrogen acylation was limited by using a
cyanomethylester of the amino acid.17,18 Given that Hecht has shown
previously that tandemly activated suppressor tRNAs, made by bis-amino acylation of pdCpA, are
capable of participating in protein synthesis, we do not need to concern
ourselves with diacylation.26-29
Before beginning the time consuming
process of protecting already synthesized materials with the appropriate
protecting groups, we needed a proof of concept that a coupling reagent would
be effective in regards to the acylation of pdCpA. We utilized a N,N�-diisopropylcarbodiimide
(DIC) coupling reagent in conjunction with our protected amino acid (Baa) and
pdCpA (Scheme 3). We investigated both Fmoc-Baa and the less bulky Boc-Baa
through this method (Boc = tert-butyloxycarbonyl).
After multiple attempts with DIC, some product (M+ + Na + H m/z 1815.49, calcd. m/z 1815.26) was detected by MALDI MS from the crude reaction
mixture. However, after analysis by analytical HPLC none of the collected
fractions containing an absorbance at 330 nm (characteristic of the presence of
C60) proved to be the correct mass to charge ratio (m/z) by MALDI MS. Since we were unable
to isolate our compound of interest, there was no way to determine whether
oxygen or nitrogen acylation had occurred. In all subsequent attempts, under
more forcing conditions, not even acylation of the free amines was observed.
Given that both the published methods and our more
aggressive coupling strategy had failed to acylate pdCpA, the reactivity of the
dinucleotide diols needed to be confirmed to determine if the lack of
reactivity is due to steric considerations of the C60 residue. In
this regard, a perfluorophenyl ester of Fmoc-glycine (Fmoc-Gly-OPfp) was
coupled to pdCpA in anhydrous DMF (Scheme 4). As expected, mono- and
di-acylation products were observed by HPLC and MALDI MS. To confirm that we
had at least some oxygen acylation, a portion of the reaction mixture was
shaken with a 5% bicarbonate solution thereby cleaving the ester linkages but
leaving amide bonds intact. The change in the chromatograms before and after
the bicarbonate treatment confirmed that a large degree of esterification had
occurred (Figure 1). It is worth noting that no triacylated products were
observed in the mass spectrum of the crude reaction mixture and
very little diacylated product remained after the bicarbonate treatment. After
witnessing the ease with which the acylation with an unhindered amino acid
should occur with coupling reagents, it became clear that we would have to find
some other way of bringing these two molecules together.
Figure 1: The HPLC chromatograms (blue
is before bicarbonate treatment and pink is after) of the reaction mixture
showing major and minor products from the coupling between pdCpA and
Fmoc-Gly-OPfp.
Conclusions
Our investigation into the acylation pdCpA
with the fullerene amino acid (Baa) led us to the conclusion that this
particular fullerene amino acid does not react with pdCpA. Even under rigorous
coupling conditions with a DIC coupling reagent, very little product was
observed. However, pdCpA is easily acylated with other less hindered amino
acids as we have demonstrated with a perfluorphenyl ester of glycine. Based on
our results we decided to pursue alternate strategies for acylation of pdCpA
with fullerene amino acids. There are two possibilities: 1) to attach C60
as the final step after the acylation of pdCpA or 2) utilize a fullerene amino
acid with a more flexible side chain such as lysine.30 Both of these
strategies require a new route fullerene derivatized amino acids.
Experimental
Nucleoside
precursors and phosphoramidte coupling reagents were purchased from Chem-Impex
International, Inc., unless otherwise specified, and used without further
purification. C60 fullerene (99.5% purity) was purchased from MER
Corp. and was purified chromatographically with toluene prior to use to remove
trace oxides. All other chemicals and reagents were purchased from
Sigma-Aldrich. All solvents were dried and distilled prior to use using standard
techniques unless otherwise specified. Products were purified by flash column
chromatography on silica gel (230 - 400 mesh).
1H
NMR spectra were recorded on Bruker Avance 400 and 500 MHz spectrometers. MALDI
measurements were performed on Bruker Reflex or Autoflex with TOF detection.
DCTB (2-[(2E)-3-(4-tert-butylphenyl)-2-methylprop-2-enylidene]malononitrile)
and elemental sulfur were used as the matrices to characterize fullerene
derivatives by MALDI/TOF (negative mode, M·- ionization). α-Cyano-4-hydroxycinnamic
acid (CHCA) was used as the MALDI matrix for all other compounds. UV-visible
spectra were recorded on a Varian Cary 5000 spectrometer. High performance
liquid chromatography (HPLC) was performed on a Varian ProStar instrument using
a Varian C18 column (150 x 4.6 mm) for reaction monitoring
and a Varian
Dynamax C18 column (250 x 21.6 mm) for purification.
The fullerene amino acid (Baa)24 and the hybrid dinucleotide (pdCpA)23 were synthesized according to literature methods. The phosphates of pdCpA were shielded through ion exchange with tetrabutylammonium (TBA) ions. The resin was prepared by packing a column with a cationic exchange resin (Dowex 50Wx8, 200 mesh) and flushing it with tetrabutylammonium hydroxide (TBA+ -OH, 1%, ~ 250 mL) until the eluent was basic. Next the column was flushed with deionized water until the eluent was neutral again. The nucleotide was stirred with the exchanged resin as an aqueous mixture (~ 5 mL resin/100 mg pdCpA, 5 � 10 min) in a round bottom flask in an ice bath, after which the resin was filtered out. The ion exchanged nucleotide [(Bu4N)3∙pdCpA] was lyophilized to dryness and stored as a fluffy, white powder. Stock nucleotide solutions (50 - 60 mM) were prepared in anhydrous dimethylformamide (an. DMF) as needed. 1H NMR showed a TBA to pdCpA ratio of 3.3 to 1. Concentrations of the stock solutions were determined by UV-visible spectroscopy (e260nm = 23,000 cm-1M-1).
Fmoc-Baa-OCH2CN
(1)
Fmoc-Baa (125 mg, 0.1062 mmols) and triethylamine (TEA, 29.6 mL, 0.2125 mmols) were dissolved in DMF (50 mL). The Baa solution was added by syringe to an evacuated 100 mL round bottom flask fit with a stir bar and septa. Chloroacetonitrile (ClCH2CN, 20.2 mL, 0.3189 mmols) was added to the round bottom by syringe and the reaction was stirred under an Ar atmosphere. After 24 h the reaction mixture was diluted with dichlormethane (DCM, 150 mL), washed with sodium bisulfate (0.5 M, 150 mL, 2x) and then brine (150 mL, 1x). The organic phase was then dried over sodium sulfate, filtered, and concentrated under reduced pressure. The concentrate was then applied to a column of silica gel and the cyanomethylester of Baa was eluted with 1:1 ethyl acetate:hexane. Yield: 116.2 mg, 90%. 1H NMR (400 MHz, CDCl3): d 7.69 (2H, m), 7.50 (2H, m), 7.33 (2H, m), 7.25 (3H, m), 6.97 [2H, d, J(H-H) = 7.3 Hz), 6.73 [2H, d, J(H-H) = 7.76 Hz], 5.15 (1H, s), 4.85 (1H, m), 4.76 [2H, d, J(H-H) = 15.50 Hz), 4.66 [2H, d, J(H-H) = 15.50 Hz), 4.40 (1H, m), 4.28 (1H, m), 4.13 (1H, m), 3.73 (2H, m), 3.54 (3H, m), 3.28 (1H, m), 3.02 (2H, m), 2.52 (1H, m). MALDI m/z (calcd.): (M·)- 1215.26, (1215.22).
Carbodiimide coupling
of pdCpA and Fmoc-Baa (2)
To a 1 mL conical vial with a spin vane was added 1-hyroxybenzotriazole hydrate (HOBt hydrate, 1.1 mg, 0.008 mmols), (Bu4N)3∙pdCpA stock solution (100 mL, 55 mM, 0.0055 mmols), Fmoc-Baa stock solution (113 mL, 58 mM, 0.0066 mmols) both prepared in an. DMF, and N,N�-diisopropylcarbodiimide (DIC, 1.1 mL, 0.00715 mmols). The reaction was allowed to stir for 5 h after which 2 mL of the reaction mixture was diluted to 50 mL with 1:1 acetonitrile (MeCN):0.1% TFA and centrifuged (4400 RPM, 2 min.). Analytical HPLC [0.1% aq. TFA (100 � 0%) and acetonitrile (0 � 100%) over 50 min., detection at 260 nm and 330 nm] showed only one fraction with absorbance at both 315 and 260 nm, which was found to be HOBt. No product was isolated. MALDI m/z (calcd.): (M + H + Na)- 1815.49, (1815.26).
Synthesis of
pdCpA-Fmoc-Gly (3) and
pdCpA-(Fmoc-Gly)2 (4)
(Bu4N)3∙pdCpA (100 mL, 55 mM in an. DMF, 0.0055 mmols) was added
to a perfluorophenyl ester of Fmoc-glycine solution in DMF (100 mL, 55 mM in an. DMF, 0.0055 mmols) in a
conical vial fit with a spin vane. The reaction was stirred at room temperature
under an Ar atmosphere for 3 h after which 2 mL
of the reaction mixture was diluted to 50 mL
with 1:1 acetonitrile (MeCN):0.1% TFA and centrifuged (4400 RPM, 2 min.). Analytical
HPLC [0.1% aq. TFA (100 � 0%) and acetonitrile (0 � 100%) over 50
min., detection at 214 nm and 260 nm] revealed two major products and almost no
starting materials remaining. Appropriate fractions were pooled and
characterized by MALDI MS using a CHCA matrix. MALDI m/z (calcd.): (M + 2H)- mono-acylated 915.16 (914.85),
di-acylated 1194.58 (1193.94).
Acknowledgments This work was
supported by the Robert A. Welch Foundation (C-0002). The authors declare no
competing financial interests.
References
1. Maggini,
M., Scorrano, G., Bianco, A., Toniolo, C., Sijbesma, R. P., Wudl, F., Prato, M.
J. Chem.
Soc. Chem.
Commun., 1994, 305.
2. Prato, M., Bianco, A., Maggini, M., Scorrano, G., Toniolo, C., Wudl, F. J. Org. Chem., 1993, 58, 5578.
3. Vol΄pin, M. E., Parnes, Z. N., Romanova, V. S. Russ. Chem. Bull., 1998, 47, 1021.
4. Yang,
J., Wang, K., Driver, J., Yang, J., Barron, A. R. Org. Biomol. Chem., 2007, 5, 260.
5. Andreeva,
I., Petrukhina, A., Garmanova, A., Babakhin, A., Andreev, S., Romanova, V.,
Troshin, P., Troshina, O., DuBuske, L. Fuller. Nanotub. Car. N., 2008, 16, 89.
6. Yang, J., Alemany, L. B., Driver, J., Hartgerink, J. D., Barron, A. R. Chem. Eur. J., 2007, 13, 2530.
7. Pellarini,
F., Pantarotto, D., Da Ros, T., Giangaspero, A., Tossi, A., Prato, M. Org. Lett., 2001, 3, 1845.
8. Bianco,
A., Da Ros, T., Prato, M., Toniolo, C. J.
Peptide Sci., 2001, 7, 208.
9. Pantarotto, D., Tagmatarchis, N., Bianco, A., Prato, M. Mini-Rev. Med. Chem., 2004, 4, 805.
10. Borman, S. Chem. Eng. News, 2007, 85, 13.
11. Str�mgaard,
A., Jensen, A. A., Str�mgaard, K. ChemBioChem, 2004, 5, 909.
12. Hecht, S. M., Alford, B. L., Kuroda, Y., Kitano, S. J. Biol. Chem., 1978, 253, 4517.
13. Heckler, T. G., Zama, Y., Naka, T., Hecht, S. M. J. Biol. Chem., 1983, 258, 4492.
14. Heckler, T. G., Chang, L.-H., Zama, Y., Naka, T., Chorghade, M. S., Hecht, S. M. Biochemistry, 1984, 23, 1468.
15. Roesser, J. R., Chorghade, M. S., Hecht, S. M. Biochemistry, 1986, 25, 6361.
16. Bain, J. D., Glabe, C. G., Dix, T. A., Chamberlin, A. R. J. Am. Chem. Soc., 1989, 111, 8013.
17. Noren, C. J., Anthony-Cahill, S. J., Griffith, M. C., Schultz, P. G. Science, 1989, 244, 182.
18. Robertson, S. A., Ellman, J. A., Schultz, P. G. J. Am. Chem. Soc., 1991, 113, 2722.
19. Ye, S., Berger, A. A., Petzold, D., Reimann, O., Benjamin, M., Koksch, B. Beilstein J. Org. Chem., 2010, 6, No. 40.
20. Muranaka, N., Miura, M., Taira, H., Hohsaka, T. ChemBioChem, 2007, 8, 1650.
21. Fahmi, N. E., Golovine, S., Wang, B., Hecht, S. M. Carbohydr. Res., 2001, 330, 149.
22. Hohsaka, T., Kajihara, D., Ashizuka, Y., Murakami, H., Sisido, M. J. Am. Chem. Soc., 1999, 121, 34.
23. Zhu,
X.-F., Scott, A. I. Nucleos. Nucleot. Nucl., 2001,
20, 197.
24. Yang, J.,
Barron,
A. R. Chem. Commun., 2004, 2884.
25. Ninomiya, K., Kurita, T., Hohsaka, T.,
Sisido, M. Chem. Commun., 2003,
2242.
26. Wang, B. X., Zhou, J., Lodder, M., Anderson III, R. D., Hecht, S. M J. Biol. Chem., 2006, 281, 13865.
27. Duca, M., Chen, S. X., Hecht, S. M. Org. Biomol. Chem., 2008, 6, 3292.
28. Duca, M., Chen, S. X., Hecht, S. M. Methods, 2008, 44, 87.
29. Maloney, D. J., Ghanem, N., Zhou, J., Hecht, S. M. Org. Biomol. Chem., 2007, 5, 3135.
30. Strom, T. A., Barron, A. R. Chem. Commun., 2010, 4764.
This website is optimized for the last versions of Internet Explorer (V. 7 or higher) and Firefox. We therefore advise to download (or upgrade your internet browser to) IE 7 or Firefox. All rights reserved to The All Results Journals (c).
To help promote The All Results Journals you can now download our poster and display it in your library, common room, office or laboratory.
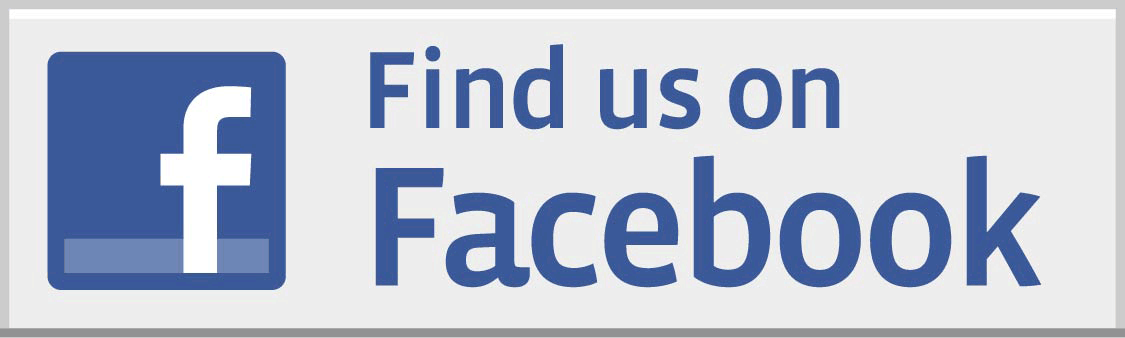
